- Yawning is a behaviour to which little
research has been devoted. However, its purpose
has not yet been demonstrated and remains
controversial. In this article, we propose a new
theory whereby, when the default mode network is
active, yawning exteriorizes a process of
switching to the attentional system through its
capacity to increase circulation of
cerebrospinal fluid, thereby increasing
clearance of somnogenic factors (prostaglandin
D(2), adenosine and others) accumulating in the
CSF.
- Le
bâillement, le mode par défaut et
le liquide cérébro-spinal
Walusinski O
- Pourquoi
bâillons-nous ? Revue des théories
d'Hippocrate à nos jours Walusinski
O
-
Sleep-inducing
factors
- García-García F,
Acosta-Peña E, Venebra-Muñoz A,
Murillo-Rodríguez E.
- CNS Neurol Disord Drug
Targets.
2009;8(4):235-244
-
- Connectivity
gradients between the default mode and attention
control networks
- Anderson JS, Ferguson MA, Lopez-Larson
M,
- Yurgelun-Todd D.
- Brain Connect.
2011;1(2):147-157
-
- A
conjecture as to the physiological origins of
yawning. Dolkart KM
-
- Provine writes in his most recent book:
"The hydraulic brain message produced by
coughing, sneezing, yawning, and other acts may
produce unappreciated secondary behavioral
consequences, including alterations of
attention, mood, or state of arousal"
(Provine, 2012). We propose to develop this
concept concerning yawning.
-
- Yawning is a stereotyped and often
repetitive motor act characterized by gaping of
the mouth accompanied by a long inspiration of
breath, a brief acme, and then a short
expiration of breath. Simultaneous stretching
and yawning is known as pandiculation, which is
not merely a simple opening of the mouth but a
complex, coordinated movement bringing together
a flexion followed by an extension of the neck
and a wide dilatation of the pharyngolarynx with
strong stretching of the diaphragm and
anti-gravity muscles (Provine, 1986; 2005).
Yawning is observed in cold-blooded and
warm-blooded vertebrates, from reptiles with
rudimentary "archaic" brains to human primates,
in water, air and land environments. Ethologists
agree that almost all vertebrates yawn. Yawning
is morphologically similar in reptiles, birds,
mammals and fish (Baenninger, 1987; Walusinski
and Deputte, 2004). These behaviors may be
ancestral vestiges maintained throughout
evolution with little variation, bearing witness
to the early phylogenetic origins of yawning.
Like any phylogenetically old behavior, yawning
can be observed early in ontogeny, i.e. at 12
weeks of fetal life (Walusinski, 2012).
-
- Yawning is involuntary and only humans seem
capable of altering its occurrence for cultural
or social reasons. It is highly stereotypical
because no environmental input changes the
sequence of movements. Behavioral and
neurophysiological studies provide converging
evidence that yawning occurs preferentially
during rest, periods of drowsiness and awakening
or is associated with hunger and satiety. The
frequency of yawning has a distinctive circadian
distribution and occurs most frequently before
and after sleep, i.e. during periods of lower
levels of vigilance and alertness. Furthermore,
the yawning rate correlates with the
individual's subjective feeling of drowsiness
and adjusts to individual circadian rhythms
(Baenninger et al., 1996; Giganti et al., 2010;
Provine, 2005).
-
- Nevertheless, the purpose of this behavior
remains controversial. As outlined by Guggisberg
et al.: "The existing scientific literature
on yawning is characterized by a relative
abundance of theoretical considerations and
hypotheses which contrasts with a scarcity of
experimental data". But should we be
satisfied with their conclusion? "The
argument that from an evolutionary perspective,
yawns must have a 'primitive' physiological
function arises from imprecise reasoning"
(Guggisberg et al., 2010; 2011). Concerning the
most recent theories, the findings of Gallup et
al. are consistent with a brain cooling
hypothesis, whereas Thompson links cortisol
levels with yawning episodes (Gallup and
Eldakar, 2012; Thompson, 2011). Bertolucci
suggests that yawning and pandiculation might
have an auto-regulatory role regarding the
locomotor system, i.e. to maintain the animal's
ability to express coordinated and integrated
movement by regularly restoring and resetting
the structural and functional equilibrium of the
myofascial system (Bertolucci, 2011). In any
case, it is certain that yawning opens the
Eustachian tube, inflating the lungs and thus
spreading the surfactant of the alveoli; it also
signals drowsiness and boredom (Baenninger,
1997). Robert Provine asks with humor: "Does
the flamboyant act of yawning, spontaneous or
contagious, serve a function? Or is it much ado
about nothing?" but concludes: "Yawning is a
response to and facilitator of change in
behavioral or physiological state" (Provine,
2012). In their landmark 1963 study, Ferrari et
al. concluded, "Stretching and yawning are two
physiological acts that might be considered as
an effort of the body to delay the onset of
sleep and a mechanism to reinforce wakefulness
after sleep". Inspired by these ideas, we want
to highlight how yawning, a daily behavior, may
be the visible aspect of a homeostatic process
during the shift between the default-mode
network (DMN) and the attentional network. This
process may involve the cerebrospinal fluid
clearance of a somnogenic purine nucleoside,
adenosine.
-
- Brain homeostasis: a brief
overview
- On one hand, survival depends on the
maintenance of the body's physiology within an
optimal hemeostatic range; one the other, the
principal function of the central nervous system
(CNS) is to adapt an organism's behavior to
changes in the environment. These statements
underscore the need for brain homeostasis,
including the regulation of synapse elimination,
neurogenesis and neuronal surveillance.
Homeostasis, the maintenance of optimal internal
conditions, is achieved through a complex set of
physiological and behavioral responses to
external and internal stimuli. Body temperature,
blood pressure, and nutrient and energy levels
all have precise homeostatic ranges. When the
internal milieu is challenged, physiological
responses are initiated in order to defend the
homeostatic range. The ultradian wake-sleep
cycle, a cerebral function by and for the brain,
is the basic temporal module of physiological
regulation underlying the behavioral continuum
and responds also to CNS homeostatic processes.
Indeed, it has been posited that a critical
function of sleep is synaptic renormalization
following a net increase in synaptic strength
during waking periods (Vyazovskiy and Tobler,
2012; Born and Feld, 2012).
-
- Arousal and attention, rest and
sleepiness
- Maintaining attention for more than a few
seconds is essential for mastering everyday
life. Sustaining attention is a multicomponent,
nonunitary mental faculty, involving a mixture
of sustained/recurrent processes subserving
task-set/arousal maintenance and transient
processes subserving the target-driven
reorienting of attention. For this purpose, the
brain is organized into a collection of
specialized functional networks that flexibly
interact to support various cognitive functions
like the unrivaled human attentional control. On
one hand, the facility with which humans perform
and shift among a wide variety of cognitive
tasks seems to indicate a mechanism for entering
into a task-dependent mode. On the other, a set
of brain regions, namely the default-mode
network, is active when the mind is not engaged
in specific behavioral tasks (vigilant
attention) or during sleep onset. This network
has low activity during focused attention on the
external environment.
-
- Attentional networks
- Central to many behavioral functions,
attention is one of the oldest and most pivotal
issues in psychological science. William James
(1842-1910) was the first to write about its
multiplicity (James, 1894). Cortical and
subcortical networks mediate different aspects
of attention. Without the modulatory influence
of subcortical areas, the brain would not attend
effectively. On the basis of detailed
neuroanatomical, electrophysiological and
neurochemical studies in animals, as well as
human neuroimaging data, researchers have
identified large-scale cortical-subcortical
circuits, including feedback loops and
re-entrant connections, that subserve different
aspects of attention and working memory.
Subcortical circuits, such as the
fronto-striato-thalamo-cortical loops have been
found to play an important role. The right
dorsolateral prefrontal cortex acts in an
executive capacity, monitoring performance or
arousal levels and regulating them accordingly,
in conjunction with the anterior cingulate
cortex (ACC) or other midline frontal
structures. The right inferior parietal region
participates equally in both endogenous and
exogenous alerting. The left hemisphere has been
associated with linking temporal and spatial
information, and the specific presentation of
warning signals. The pulvinar, superior
colliculus, superior parietal lobe,
temporoparietal junction, superior temporal lobe
and frontal eye fields are activated as an
orienting network. Authors have proposed a
conflict-monitoring model suggesting that the
ACC engages the dorsolateral prefrontal cortex
(DLPFC), which might be mediated by the locus
coeruleus and dopaminergic sites in the ventral
tegmental area. These findings suggest that the
DLPFC might support heteromodal conflict
resolution, whereas the ACC could be specific to
the resolution of response conflict (fig. 1).
Although disparate modules of attention
constitute an important model, the exact nature
of these networks and the degree to which they
are independent is still not clear (Harris and
Thiele, 2011; Raz and Buhle, 2006; Zhang et al.,
2008).
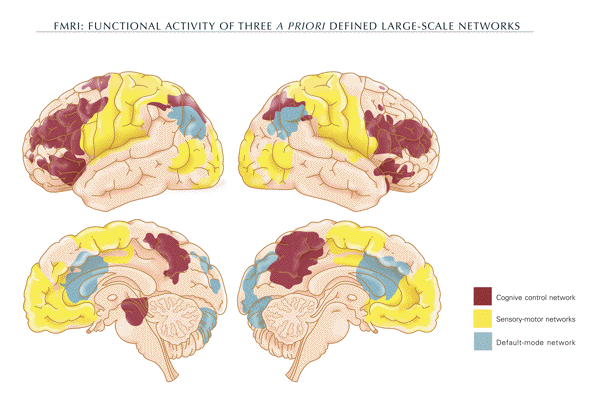
-
- The default mode network
- A set of brain regions has been identified
via functional magnetic resonance imaging. These
regions are collectively termed the
"default-mode network" (DMN) because their
pattern of spontaneous physiological activity is
detectable during the normal resting state.
Areas collectively activated during the default
mode state involve a set of midline brain
structures, including the anterior cingulate
cortex and the ventral and dorsal medial
prefrontal cortex. Moreover, the
precuneus/posterior cingulate cortex plays a
pivotal role (fig. 1) (Raichle et al., 2001;
Raichle and Snyder, 2007).
-
- DMN is characterized by high activity when
the mind is not engaged in specific behavioral
tasks and low activity during focused attention
on the external environment. The function of the
DMN has been attributed to introspection,
self-awareness, and theory of mind judgments,
and some of its regions are involved in episodic
memory processes. In a word, DMN function
postulates its involvement in self-referential
processing and thought (i.e., internal
mentation, daydreaming, etc. ), which is
typically in opposition to externally oriented
goal-directed cognition. Thus, emerging
task-relevant effects clearly support the
conclusion that DMN synchronous suppression is
functionally important for successful operation
of certain cognitive processes, such as focused
attention and working memory. Such task-based
deactivations show an antagonism with focused
attention. This anti-correlated organization
appears to be a fundamental property of the
central nervous system. (Anticevic et al., 2012;
Dosenbach et al., 2007; Dosenbach et al., 2008;
Fox et al., 2009; Tang et al., 2012).
- Oakley and Halligan have suggested that a
deviation from the normal default mode activity
might provide a neural signature of hypnosis
(Oakley and Halligan, 2009). Altered activation
of the default network has been reported in
functional imaging studies of patients with
neuropsychiatric disorders such as dementia,
schizophrenia, epilepsy, anxiety and depression,
autism and attention deficit/hyperactivity
disorder (Buckner et al., 2008; Broyd et al.,
2009). Unfortunately, none of these studies
mention the patients' yawning.
-
- Sleep and arousal
- During World War I, a pandemic of
encephalitis lethargica swept the globe. This
presumed viral infection of the brain caused a
profound and prolonged state of sleepiness,
followed by parkinsonism, in most individuals.
An Austrian neurologist, Constantin von Economo
(1876-1931), reported that this state of
prolonged sleepiness was due to injury to the
posterior hypothalamus and rostral midbrain. He
also recognized that one group of individuals
infected during the same pandemic instead had
the opposite problem: a prolonged state of
insomnia marked by salvos of yawning that
occurred with lesions of the preoptic area and
basal forebrain. Based on his observations, von
Economo predicted, with acute foresight, that
the region of the hypothalamus near the optic
chiasm contains sleep-promoting neurons, whereas
the posterior hypothalamus contains neurons that
promote wakefulness (von Economo, 1931).
-
- Currently, we know that the ventrolateral
preoptic nucleus contains GABAergic and
galaninergic neurons that are active during
sleep and necessary for normal sleep. The
posterior lateral hypothalamus contains
hypocretin neurons that are crucial for
maintaining normal wakefulness. A model was
proposed by Clifford B. Saper in which wake- and
sleep-promoting neurons inhibit each other,
which results in stable wakefulness and sleep.
Curiously, the thalamocortical system is
activated in both wakefulness and rapid eye
movement (REM) sleep. One key distinction is the
activity in distinctive hypothalamic branches of
the ascending arousal system. The firing
differences in the cholinergic and monoaminergic
ascending arousal systems characterize and
probably regulate the production of the
different behavioral states (Fuller et al.,
2006; Koike et al., 2011; Larson-Prior et al.,
2011; Saper et al., 2001; 2005).
-
- G. William Domhoff's theory suggests that a
neural substrate for dreaming may be based on a
subsystem of the waking default network, which
is active when the mind is wandering,
daydreaming, or simulating past or future events
and partially active during REM sleep. The
sudden switch to dreamlike thinking, whether at
sleep onset, during the drowsiness of a slow
morning awakening, or in brief episodes of
relaxed waking thought, suggests that the
transition to dreaming can be rapid (Domhoff,
2011). When a specific constellation of neural
regions is activated in a context where there is
no engagement with the external world, it is
plausible that, as an On-off switch, an
involuntary behavior serves to rapidly reverse
the state towards engagement with the external
world. Yawning, a homeostatic process involving
circadian variations in vigilance and emotion
may be such a behavior. Indeed, yawning
manifests a parasympathetic stimulation during
the balancing of adrenergic and cholinergic
homeostasis in the autonomic nervous system
(Jackson et al., 2011).
-
- Neurophysiology of yawning
- Several clinical and pharmacological
arguments indicate that yawning involves the
hypothalamus, particularly the paraventricular
nucleus (PVN), the brainstem and the cervical
medulla. PVN is an integration centre between
the central and peripheral autonomic nervous
systems. It is involved in numerous functions
ranging from feeding, metabolic balance, blood
pressure and heart rate, to sexual behavior and
yawning. In particular, a group of oxytocinergic
neurons originating in this nucleus and
projecting to extra-hypothalamic brain areas
(e.g. hippocampus, medulla oblongata, and spinal
cord) controls yawning. Oxytocin activates
cholinergic neurotransmission in the hippocampus
and the reticular formation of the brainstem.
Acetylcholine induces yawning by acting as an
agonist for the muscarinic receptors of muscles
triggered by the motor nuclei of the Vth, VIIth,
IXth, Xth, and XIIth cranial nerves, the phrenic
nerves (C1&endash;C4) and the motor supply to
the intercostal muscles (Argiolas and Melis
1998; Collins and Eguibar, 2010).
-
- The activating system consists of neurons
located in the midbrain reticular formation (the
reticular activating system, RAS) projecting to
the thalamus and to the cortex. An intrinsic
function of RAS is its participation in
responses such that alerting stimuli
simultaneously activate thalamocortical systems,
as well as postural and locomotor systems, in
order to enable an appropriate behavior (fight
versus flight). The neurons are mostly
noradrenergic and particularly concentrated in
small nuclei like the locus coeruleus, having
widespread projections to forebrain areas and to
virtually all brain regions. Locus coeruleus
activity varies primarily with the state of
vigilance, and has a role in regulating
different types of cognitive abilities during
alertness. The thalamic nucleus and the PVN
belong to a neural loop circuitry sending and
receiving histaminergic projections from the
tuberomammillary nucleus, and noradrenergic
projections from the locus coeruleus. The basal
ganglia, as a rule, are highly interconnected
with the pedunculopontine tegmental nucleus
(PPN). The motor function of PPN is to control
postural muscle tone. It also plays a role in
regulating the sleep-wake cycle and is a
limbic-motor interface for reward predictions.
Taken together, these characteristics suggest
that visceral and musculoskeletal sensory
pathways are connected to the same subcortical
structures involved in arousal and attention
mechanisms. From this perspective, yawning
triggers the stimulation of the locus coeruleus
through feedback from musculoskeletal and
visceral sensory inputs. For example, during the
powerful contraction caused by yawning, the
spindles of the masticatory muscles (masseter,
temporalis, pterygoids), which have receptors
that respond to stretching, send stimuli via
afferent nerves of the Ia category, which are
located in the mesencephalic root of the
trigeminal nerve (ascending visceral
parasympathetic pathway). With the motor neurons
of the same muscles these nerves form a
monosynaptic link. This is the basis of the
masseteric reflex. These nerves have projections
on RAS and the locus coeruleus which are
anatomically close to the nucleus of the
trigeminal nerve. Through the massive
contraction of the masseteric muscles, yawning
stimulates those structures responsible for
cortical activation (Barbizet, 1958; Saper,
2013; Walusinski, 2006).
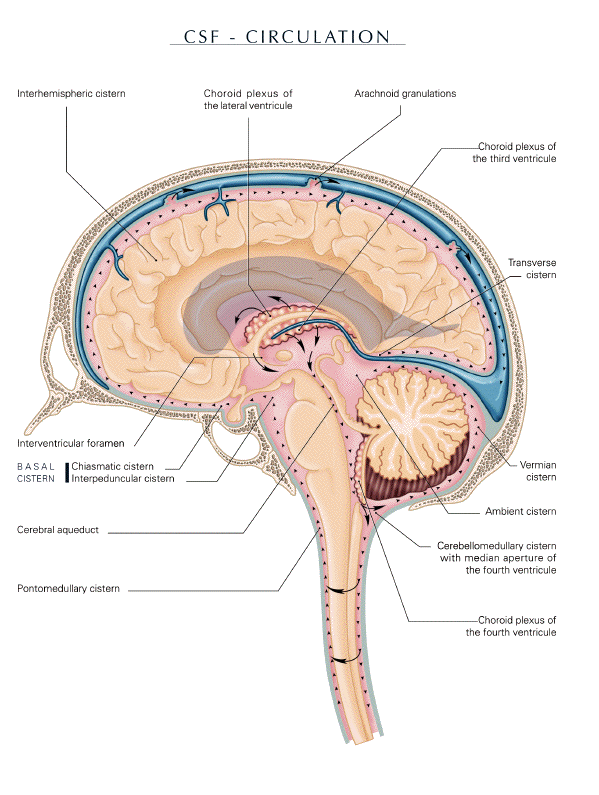
-
- Cerebrospinal fluid system homeostasis
and yawning
- The clustering of arachnoid villi along the
sagittal sinus forms, known as "Pacchioni
granulations", were first described in 1705, by
the Italian scientist Antonio Pacchioni
(1665-1726), as "peculiar wart like
excrescences". But he failed to ascribe the
right function to them (Brunori et al., 1993;
Pacchoni, 1705). In 1768, Domenico Cotugno
(1736-1822) clearly evoked the presence of fluid
surrounding the nervous system and its
circulation: "The whole space between the
dura mater and the medulla is always filled; not
by the medulla which is more turgescent in the
living, nor by a water vapour (as this yet
obscure substance is suspected of being by noted
authors); but by water, similar to that about
the heart which the pericardium holds, which
fills the ventricles of the brain and the
labyrinths of the ear, as well as the other
cavities of the body inaccessible to air"
(Cotugno, 1768). François Magendie
(1783-1855) confirmed this finding in 1825
through comparative observations of animals and
humans (Magendie, 1825). However, the initial
experimental work on cerebrospinal fluid (CSF)
outflow was performed during the landmark study
of von Axel Key (1832-1901) and Gustaf Retzius
(1842-1919) in 1876. They showed that lymphatic
drainage has at least a potentially significant
role in the outflow of CSF. A growing body of
recent evidence not only suggests the importance
of both arachnoid granulations and lymphatic
capillaries, but also that transependymal
passage plays a role in CSF outflow (fig. 2)
(Key and Retzius, 1876; Magendie, 1825;
Stahnisch, 2008; Woollam, 1957).
-
- The sum of the intracranial volumes of
brain, blood, and CSF is assumed to be constant.
CSF motion is therefore caused by the change of
the cerebral blood volume due to the difference
between arterial inflow and venous outflow and
the movements associated with breathing that
cause pressure variations in the ventricular
system. Each deep inhalation is followed be an
increase in CSF flow rate in the fourth
ventricle. CSF first gains access to drainage
sites along the base of the cranium, and as
intracranial pressure increases, CSF flow may
move to the subarachnoid spaces along the
convexity of the brain to absorption sites
associated with cranial venous sinuses.
Variations in blood volume within the rigid
skull are compensated for by displacement of the
CSF, brainstem, and spinal cord which act like a
piston in the foramen magnum, balancing the
intracranial volume. During expiration,
increasing intracranial vascular pressure leads
to compensatory caudal movement; during
inspiration the pressure decreases and the
balancing displacement must occur in the cranial
direction (fig. 2) (Kapoor et al, 2008; Maier et
al, 1994; Schroth and Klose, 1992).
-
- Jaw kinematics and inhalation alter
intracranial circulation. The consequences of
yawn variants involving jaw and airway
maneuvers, i.e. closed-nose yawn or
clenched-teeth yawns, suggested by Provine, or
the Valsalva maneuver, commonly used to
designate any forced expiratory effort against a
closed airway, are the same as for physiological
yawning (Provine, 2012). Indeed, all behavior
inducing a cervical compression of the jugular
immediately increases the cerebrospinal fluid
pressure. Therefore, wide mouth opening, such as
yawning or variants, may have the same effect by
stretching the omohyoid muscles and thus
squeezing the jugular veins. Hence, Friedrich H.
Lepp (1982) described jaw kinematics as follows.
Jaw movements activate the pterygoid
musculovenous pump, located in the upper part of
the anterior parapharyngeal space, known as the
prestyloid parapharyngeal space. As a result,
this pump, also known as the paratubal pump, can
impact the mechanism of venous blood flow out of
the endocranium, mainly via the plexus venosus
foraminis ovalis. The pterygoid cistern, a
component of this pump, corresponds to the
cavernous part of the pterygoid plexus. It is an
extracranial extension of the cavernous sinus
and passes through the foramen ovale. It plays
an important role as an intermediary station of
acceleration for return blood flow from the
brain (Bouyssou and Tricoire, 1985; Patra et
al., 1988). Lepp (1982) noted that it would be
reasonable to consider jaw kinematics together
with the lateral pterygoid muscle as a venous
trigger, given that they act as the starter for
the alternating musculovenous pumping action
that takes place in the cavernous part of the
pterygoid plexus. This pumping action is
particularly efficient during isolated yawning,
especially when the mouth reaches its maximum
opening. However, Lepp emphasized that yawning
itself, and pandiculation even more so, is often
merely the initiation of a musculovenous motor
chain reaction, which extends to the limbs and
the entire skeletal musculature as tonic waves
propagated in the rostrocaudal direction to the
ends of the fingers and toes. It would thus
appear that the large inhalation and maximum
opening of the mouth accelerate the circulation
of CSF (Nitz et al., 1992).
-
- Research into the hormonal factors that
induce sleep has been conducted for nearly 100
years. In 1912, René Legendre (1880-1954)
and Henri Piéron (1881-1964) demonstrated
the presence of a hypnogenic factor in the CSF,
which accumulates during the waking state. They
took CSF samples of sleep-deprived dogs and
infused them into the brains of normal dogs. The
recipient dogs soon fell asleep. Thus these two
authors became the first to demonstrate the
presence of endogenous sleep-promoting
substances, but did not identify the chemical
nature of their sleep substances (Legendre and
Pieron, 1912). During the next 90 years, nearly
50 endogenous sleep substances were reported by
numerous investigators to be present in the
brain, CSF, and other organs and tissues of
mammals, although their physiological relevance
has remained uncertain in most instances.
Nevertheless, concentrations of these molecules
in CSF appear to be directly dependent upon
their rate of production in the brain. Available
evidence indicates prostaglandin PGD(2) as a
most plausible candidate. PGD(2) is produced by
lipocalin-type PGD synthase localized in the
leptomeninges, choroid plexus and
oligodendrocytes, and circulates in the
cerebrospinal fluid as a sleep hormone. During
the past several decades, the mechanism of
signal transduction has been extensively studied
by a number of investigators at the cellular
level. These studies indicate that most, if not
all hormones, cytokines, and neurotransmitters
do not penetrate the cell membrane. Instead they
are bound to specific receptors on the cell
surface, and the signals are then transmitted
through these receptors via so-called second
messengers such as cyclic AMP, Ca2, and so
forth. The mechanisms underlying sleep
regulation by PGD(2) are somewhat reminiscent of
signal transduction mechanisms at the cellular
level; namely, PGD(2) is bound to D-type
prostanoid receptors on the surface of the
meninges, followed by the transduction via the
purine nucleoside adenosine through the
adenosine A2A receptor, increasing the local
extracellular concentration of adenosine in the
basal forebrain as a paracrine sleep-promoting
molecule. Indeed, this signal is transmitted
across the leptomeninges into the brain
parenchyma towards the ventrolateral preoptic
(VLPO) nucleus of the anterior hypothalamus,
which induces sleep. Sleep-promoting neurons in
the VLPO send inhibitory signals to suppress the
histaminergic neurons in the tuberomammillary
nucleus (TMN), which contribute to arousal
through histamine H1 receptors. The neural
network between VLPO and TMN is considered to
play a key role in the regulation of sleep.
Although PGD(2) level in CSF affects the action
of D-type prostanoid receptors that promote
physiological sleep, the regulatory system of
PGD(2) clearance from the CSF is not fully
understood (Huang et al., 2007; 2011; Urade and
Hayaishi, 2011)
-
- Taking into consideration all mechanisms and
pathways helping us to understand the
significance of CSF outflow, we argue that
yawning and pandiculation may accelerate
clearance of PGD(2), thus reducing sleepiness.
They may also act on other neuromediators that
are currently unknown. There have been no
studies on the impact of adenosine level on DMN
activity but, for example, recent findings
highlight the link between astrogliosis,
dysfunction of adenosine homeostasis and seizure
generation (Aronica et al., 2013; Tachikawa et
al., 2012).
-
- Older data that initially seem to contradict
our proposal must also be taken into account.
Adenosine mediated effects on sleep-wake cycles
are site- and receptor-dependent. Indeed, in the
lateral preoptic area, a region with an
abundance of sleep-active neurons, adenosine
acting via A1 receptors induces waking by
inhibition of sleep-active neurons. On the
contrary, adenosine acting via A2A receptors
promotes sleep by stimulating sleep-active
neurons. Hence, A1 adenosine receptors may exert
a negative influence on apomorphine-induced
yawning. For example, intracerebroventricular
administration of physostigmine to rats induces
yawning dose-dependently and a selective A1
receptor agonist (N6-cyclohexyladenosine)
reduces the yawning induced. Theophylline or
caffeine, two adenosine receptor antagonists,
exert an A2 antagonist effect. Hence, it may be
possible that blocking the A2 adenosine receptor
unmasks the A1 receptor subtype and in turn,
elicits inhibition of apomorphine-induced
yawning. So, adenosine via the A1 receptor
subtype inhibits yawning while adenosine via A2A
receptors elicits yawning (Methippara et al.,
2005; Ushijima et al., 1992; Zarrindast et al.,
1995-1; 1995-2). Such results point out the need
for experimental measurements of PGD(2) levels
in the CSF, before, during and after yawning;
such data are currently lacking.
-
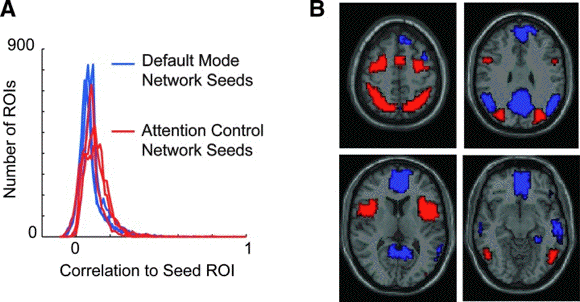
- Identification of default mode and
attention control networks.
- (A) Distribution of correlation to 6 seed
regions of interest (ROIs; 3 for each network)
for 7266 ROIs covering the gray matter.
- (B) Boundaries of the default mode (blue)
and attention control (red) networks used for
subsequent analyses.
-
- Tentative conclusion
- Changing between attention levels requires
withdrawal from DMN activity and a redeployment
to active vigilance by attentional network
processing. At present, we know little about the
neural processes responsible for this switch and
those that initiate the reset between the
attentional network and DMN. Such processes must
adapt to the contingencies of environmental
change and to the consequences for the organism
of its own effect and must maintain optimum
internal conditions. In the framework of neural
networks that govern behaviors, automatic
actions such as yawning may constitute a robust
"attractor state" in which the resting state
neural system (DMN) is readily dislodged and
another stable state allowing sustainable
attention (attentional network) is engaged.
-
- Our theory takes into account three levels
of data, in accordance with Provine and
Ferrari's proposal that yawning "might be
considered as an effort of the body to delay the
onset of sleep and a mechanism to reinforce
wakefulness after sleep". Each level is
explained by the following:
- 1) the clinical level: yawning appears when
the main source of stimulation in a person's
environment no longer sustains his or her
attention, by content or by form, i.e. yawning
appears when DMN is active and sleepiness
increases;
- 2) the network level: yawning disengages DMN
to promote the attentional network;
- 3) the molecular level: yawning accelerates
the circulation of the CSF. By this action, the
increased clearance of somnogenic substances
reduces the propensity toward sleepiness.
-
- In other words, yawning is proposed as a
homeostatic process that regulates the level of
sleep-inducing molecules and disengages DMN to
promote the attentional network. By this
proposition, we aim to open up a new avenue for
elucidating the interplay between the humoral
regulation and the neural network of yawning and
its physiological function. We are aware that
our hypothesis should be subjected to
experimental testing. Hopefully, it provides a
foundation on which to base a new class of
innovative studies on yawning.
-
- David Frederick Horrobin (1939-2003)
summarizes this perspective: "The history of
science has repeatedly shown that when
hypotheses are proposed, it is impossible to
predict which will turn out to be revolutionary
and which ridiculous. The only safe approach is
to let all see the light and to let all be
discussed, experimented upon, vindicated or
destroyed" (Horrobin, 1976).
-
-
- Acknowledgements
- We thank the revievers for their pertinent
suggestions and Maria Rosaria Melis for her
thoughtful comments in the preparation of this
manuscript (Department of Biomedical Sciences,
Neuroscience and Clinical Pharmacology Section,
University of Cagliari, Italy).
-
- Illustrations by Hélène
Badault for the author
-
- References
-
- Anticevic
A, Cole MW, Murray JD, Corlett PR, Wang XJ,
Krystal JH. 2012. The role of default
network deactivation in cognition and disease.
Trends Cogn Sci. 16:584-92.
-
- Argiolas
A, Melis MR. 1998. The neuropharmacology of
yawning. Eur J Pharmacol. 343:1-16.
-
- Aronica
E, Sandau US, Iyer A, Boison D. 2013. Glial
adenosine kinase - A neuropathological marker of
the epileptic brain. Neurochem Int. In press.
http://dx.doi.org/10.1016/j.bbr.2011.03.031.
-
- Baenninger
R. 1987. Some comparative aspects of yawning
in Betta slpendens, Homo Sapiens, Pantera leo
and Papio sphinx J.Comp. Psychol.
101:349-354.
-
- Baenninger R,
Binkley S, Baenninger M. 1996. Field
obsvations of yawning and activity in humans.
Physiol Behav. 59:421-5.
-
- Baenninger
R. 1997. On yawning and its functions.
Psychon Bull Rev. 4:198-207.
-
- Barbizet
J. 1958. Yawning. J Neurol Neurosurg
Psychiatry. 21:203-9.
-
- Bertolucci
LF. 2011. Pandiculation: nature's way of
maintaining the functional integrity of the
myofascial system? J Bodyw Mov Ther.
15(3):268-80.
-
- Born
J, Feld GB. 2012. Sleep to upscale, sleep to
downscale: balancing homeostasis and plasticity.
Neuron. 75:933-935.
-
- Bouyssou M,
Tricoire J. 1985. Experimental detection of
a cervical arousal mechanism of yawning,
enhancing intracerebral (CSF) fluid pressure. J
Dental Res. 64:721.
-
- Broyd
SJ, Demanuele C, Debener S, Helps SK, James CJ,
Sonuga-Barke EJ. 2009. Default-mode brain
dysfunction in mental disorders: a systematic
review. Neurosci Biobehav Rev.
33(3):279-96.
-
- Brunori
A, Vagnozzi R, Giuffrè R. 1993.
Antonio Pacchioni (1665-1726): early studies of
the dura mater. J Neurosurg.78(3):515-8.
-
- Buckner
RL, Andrews-Hanna JR, Schacter DL. 2008. The
brain's default network: anatomy, function, and
relevance to disease. Ann N Y Acad Sci.
1124:1-38.
-
- Collins
GT, Eguibar JR. 2010. Neuropharmacology of
yawning. Front Neurol Neurosci. 28:90-106.
-
- Cotugno
D. 1768. Dominici Cotunnii philosophiae, et
medicinae doctoris ... de ischiade nervosa
commentarius. Carpi : Ex chalcographia publici.
80p.
-
- Cotugno
D. 1988. The first description of the spinal
fluid. By Domenico Cotugno, 1775. Clin Orthop
Relat Res. 227:6-9
-
- Domhoff
WG. 2011. The neural substrate for dreaming:
is it a subsystem of the default network?
Conscious Cogn. 20:1163-74.
-
- Dosenbach
NU, Fair DA, Miezin FM, Cohen AL, Wenger KK,
Dosenbach RA, Fox MD, Snyder AZ, Vincent JL,
Raichle ME, Schlaggar BL, Petersen SE. 2007.
Distinct brain networks for adaptive and stable
task control in humans. Proc Natl Acad Sci U S
A. 104:11073-8.
-
- Dosenbach
NU, Fair DA, Cohen AL, Schlaggar BL, Petersen
SE. 2008. A dual-networks architecture of
top-down control. Trends Cogn Sci.
12:99-105.
-
- Ferrari
W, Gessa GL, Vargiu L. 1963. Behavioral
effects induced by intracisternally injected
ACTH and MSH. Ann N Y Acad Sci. 104:330-45.
-
- Fox
MD, Zhang D, Snyder AZ, Raichle ME. 2009.
The global signal and observed anticorrelated
resting state brain networks. J Neurophysiol.
101:3270-83.
-
- Fuller
PM, Gooley JJ, Saper CB. 2006. Neurobiology
of the sleep-wake cycle: sleep architecture,
circadian regulation, and regulatory feedback. J
Biol Rhythms. 21:482-93.
-
- Gallup
AC, Eldakar OT. 2012. The thermoregulatory
theory of yawning: what we know from over 5
years of research. Front Neurosci. 6;188
:1-13.
-
- Giganti
F, Zilli I, Aboudan S, Salzarulo P. 2010.
Sleep, sleepiness and yawning. Front Neurol
Neurosci. 28:42-6.
-
- Guggisberg
AG, Mathis J, Schnider A, Hess CW. 2010. Why
do we yawn? Neurosci Biobehav Rev.
34:1267-76.
-
- Guggisberg
AG, Mathis J, Schnider A, Hess CW. 2011. Why
do we yawn? The importance of evidence for
specific yawn-induced effects. Neurosci Biobehav
Rev. 35(5):1302-4.
-
- Horrobin
DF. 1976. Ideas in biomedical science
reasons for the foundation of Medical
Hypotheses. Med Hypotheses. 2:29-30.
-
- Harris
KD, Thiele A. 2011. Cortical state and
attention. Nat Rev Neurosci. 12:509-23.
-
- Huang
ZL, Urade Y, Hayaishi O. 2007.
Prostaglandins and adenosine in the regulation
of sleep and wakefulness. Curr Opin Pharmacol.
7:33-8.
-
- Huang
ZL, Urade Y, Hayaishi O. 2011. The role of
adenosine in the regulation of sleep. Curr Top
Med Chem. 11:1047-1057.
-
- Jackson
SR, Parkinson A, Kim SY, Schüermann M,
Eickhoff SB. 2011. On the functional anatomy
of the urge-for-action. Cogn Neurosci.
2:227-243.
-
- James
W. 1894. Discussion: The physical basis of
emotion. Psychological Review. 1:516-529.
-
- Kapoor
KG, Katz SE, Grzybowski DM, Lubow M. 2008.
Cerebrospinal fluid outflow: an evolving
perspective. Brain Res Bull. 77:327-34.
-
- Koike
T, Kan S, Misaki M, Miyauchi S. 2011.
Connectivity pattern changes in default-mode
network with deep non-REM and REM sleep.
Neurosci Res. 69:322-30.
-
- Larson-Prior
LJ, Power JD, Vincent JL, Nolan TS, Coalson RS,
Zempel J, Snyder AZ, Schlaggar BL, Raichle ME,
Petersen SE. 2011. Modulation of the brain's
functional network architecture in the
transition from wake to sleep. Prog Brain Res.
193:277-94.
-
- Legendre
R, Piéron H. 1912. De la
propriété hypnotoxique des humeurs
développée au cours d'une veille
prolongée. C.R. Société de
Biologie de Paris. 72:210-212.
-
- Lepp FH.
1982. Remarques sur la signification
physiologique du bâillement. Bull Group
Int Rech Sci Stomtol Odontol. 25:251-90.
-
- Magendie
F. 1825. Sur un liquide qui se trouve dans
le crâne et le canal vertébral de
l'homme et des animaux mammifères.
Journal de Physiologie expérimentale et
pathologique. 5:27-37.
-
- Methippara
MM, Kumar S, Alam MN, Szymusiak R, McGinty
D. 2005. Effects on sleep of microdialysis
of adenosine A1 and A2a receptor analogs into
the lateral preoptic area of rats. Am J Physiol
Regul Integr Comp Physiol. 289:R1715-23.
-
- Nitz
WR, Bradley WG Jr, Watanabe AS, Lee RR, Burgoyne
B, O'Sullivan RM, Herbst MD. 1992. Flow
dynamics of cerebrospinal fluid: assessment with
phase-contrast velocity MR imaging performed
with retrospective cardiac gating. Radiology.
183:395-405.
-
- Oakley
DA, Halligan PW. 2009. Hypnotic suggestion
and cognitive neuroscience. Trends Cogn Sci.
13:264-70.
-
- Pacchioni
A. 1705. Dissertatio Epistolaris de
Glandulis Conglobatis Duræ Meningis
Humanae, indeque Ortis Lymphaticis ad Piam
Meningem productis. Romae, Typis Io: Francisci
Buagni. 32p.
-
- Patra P,
Gunness TK, Robert R, Rogez JM, Heloury Y, Le
Hur PA, Leborgne J, Laude M, Barbin JY.
1988. Physiologic variations of the internal
jugular vein surface, role of the omohyoid
muscle, a preliminary echographic study. Surg
Radiol Anat. 10:107-12.
-
- Provine R.
1986. Yawning as a stereotyped action
pattern and releasing stimulus. Ethology.
72:109-122.
-
- Provine R.
2005. Yawning. American Scientist.
93:532-539.
-
- Provine R.
2012. Curious Behavior, Yawning, Laughing,
Hiccuping and beyond. Cambridge, London. The
Belknap Press of Harvard University Press.
271p.
-
- Raichle
ME, MacLeod AM, Snyder AZ, Powers WJ, Gusnard
DA, Shulman GL. 2001. A default mode of
brain function. Proc Natl Acad Sci U S A.
98:676-82.
-
- Raichle
ME, Snyder AZ. 2007. A default mode of brain
function: a brief history of an evolving idea.
Neuroimage 37(4):1083-90.
-
- Raz
A, Buhle J. 2006. Typologies of attentional
networks. Nat Rev Neurosci. 7:367-79.
-
- Saper
CB, Chou TC, Scammell TE. 2001. The sleep
switch: hypothalamic control of sleep and
wakefulness. Trends Neurosci. 24:726-31.
-
- Saper
CB, Cano G, Scammell TE. 2005. Homeostatic,
circadian, and emotional regulation of sleep. J
Comp Neurol. 493:92-8.
-
- Saper
CB. 2013. The neurobiology of sleep.
Continuum (Minneap Minn). Sleep
Disorders.1:19-31.
-
- Schroth G, Klose
U. 1992. Cerebrospinal fluid flow;
Physiology of respiration-related pulsations.
Neuroradiology 35:10-5.
-
- Stahnisch
FW. 2008. Instrument transfer as knowledge
transfer in neurophysiology: François
Magendie's (1783-1855) early attempts to measure
cerebrospinal fluid pressure. J Hist Neurosci.
17:72-99.
-
- Tachikawa
M, Tsuji K, Yokoyama R, Higuchi T, Ozeki G,
Yashiki A, Akanuma S, Hayashi K, Nishiura A,
Hosoya K. 2012. A clearance system for
prostaglandin D2, a sleep-promoting factor, in
cerebrospinal fluid: role of the
blood-cerebrospinal barrier transporters. J
Pharmacol Exp Ther. 343:608-16.
-
- Tang
YY, Rothbart MK, Posner MI. 2012. Neural
correlates of establishing, maintaining, and
switching brain states. Trends Cogn Sci.
16:330-7.
-
- Thompson
S.B.N. 2010. The dawn of the yawn: is
yawning a warning? Linking neurological
disorders. Medical Hypotheses, 75:630-633.
-
- Urade
Y, Hayaishi O. 2011. Prostaglandin D2 and
sleep/wake regulation. Sleep Med Rev.
15:411-8.
-
- Ushijima
I, Mizuki Y, Ukita T, Kaneyuki H, Inano S,
Yamada M. 1992. Behavioral effects of
dilazep on cholinergic, dopaminergic, and
purinergic systems in the rat. Pharmacol Biochem
Behav. 43:673-6.
-
- von
Economo C. 1931. Encephalitis lethargica,
its sequelae and treatment. London: Oxford
University Press. 200p.
-
- von
Key A, Retzius G. 1876. Studien in der
Anatomie des Nervensystems und des Bindegewebes.
Stockholm : In Commission bei Samson &
Wallin : Druck von P.A. Norstedt &
Söner, 1875-76. 2 vol. 220-228p.
-
- Vyazovskiy
VV, Tobler I. 2012. The temporal structure
of behaviour and sleep homeostasis. PLoS One.
7:e50677.
-
- Walusinski O,
Deputte BL. 2004. The phylogeny, ethology
and nosology of yawning. Rev Neurol (Paris).
160:1011-1021.
-
- Walusinski O.
2006. Yawning: unsuspected avenue for a better
understanding of arousal and interoception. Med
Hypotheses. 67:6-14.
-
- Walusinski
O. 2010. The Mystery of Yawning in
Physiology and Disease. Basel. Karger. Front
Neurol Neurosci 28. 160p.
-
- Walusinski
O. 2012. Fetal yawning. In Sonography. K.
Thoirs Editor. Rijeka, Croatia. Intech.
346p.
-
- Woollam
DH. 1957. The historical significance of the
cerebrospinal fluid. Med Hist. 1:91-11.
-
- Zarrindast MR,
Adeli R, Hosseini-semnani S, Sharifzadeh M.
1995. Effects of adenosine receptor agonists and
antagonists on physostigmine-induced yawning.
Eur J Pharmacol. 276:3-7.
-
- Zarrindast
MR, Fatehi F, Mohagheghi-Badi M. 1995.
Effects of adenosine agents on
apomorphine-induced yawning in rats.
Psychopharmacology (Berl). 122:292-6.
-
- Zhang
D, Snyder AZ, Fox MD, Sansbury MW, Shimony JS,
Raichle ME. 2008. Intrinsic functional
relations between human cerebral cortex and
thalamus. J Neurophysiol. 100:1740-8.
-
-
|